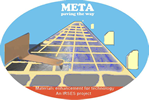
WorkPackage 1 “DNA motherboards”
(WP coordinator: Piero Morales)
WP1
Aims to exploit the self-assembling properties of biological matter to build functional nanodevices at negligible production cost. Protein based functional nanocomponents are being addressed onto specific sequences of carefully designed DNA regular nanoscopic boards. When properly designed, a molecular scale device (e.g. a molecular charge exchange system, or a scaffold for nucleic acids hybridization and reconstruction) will be self-assembled and interfaced to a more conventional solid state signal detection system built by highly advanced nanolithographic techniques. The DNA architecture that we work on is a simple square flat structure having size below 100 nm, acting as a “mother board” for self-assemblage of the protein based “plug-in components”.
Different methods for selfassemblage of the motherboards have been explored while the efficiency of aptameric DNA based “connectors” for the plug-in components are being studied. Connection to the solid state substrate and transducers is effected by electron-beam fabricated anchoring nanodots made of gold or other materials that possess selective properties of adhesion with respect to particular peptides conjugated to the motherboards. Different molecular groups have been plugged in onto our DNA motherboards, while anchoring dots arranged in the desired geometry, with the desired size and thickness have been fabricated, and the first nanometrically precise dockings have been obtained.
Objectives
Brief description: Aim of this work package is to exploit the self-assembling properties of biological matter to build functional nanodevices at negligible production cost. Protein based functional nanocomponents will be addressed onto specific sequences of carefully designed DNA regular nanoscopic grids. When properly designed, a molecular scale device (e.g. a molecular charge exchange system, or a scaffold for nucleic acids hybridization and reconstruction) will be self-assembled and interfaced to a more conventional solid state signal detection system built by highly advanced nanolithographic techniques. The DNA architectures that we will work on are simple 3xn grids (1<n<4) with element size of 20 nm, acting as “mother boards” for self-assemblage of the protein based “plug-in components”.
In general terms, we wish to couple the power of advanced nanolithography on solid state materials to the self-assembling properties of biological matter with the aim of establishing a fabrication technology for extremely specific future nanodevices at negligible production cost. More specifically, the already demonstrated properties of DNA to self-assemble in highly ordered planar and three dimensional nanoarchitectures will be used to address specific protein based nanomaterials onto predesigned locations of a DNA ordered structure. Owing to a suitable design of the DNA grid sequences on the one side, and to properly selected specific surface adhesive peptides on the other, a molecular scale device will be self-assembled and interfaced to a more conventional solid state nanoelectronic signal detection system built by advanced nanolithographic techniques. ADNA simple 3×3 square gridwith element size of 20 nm, will thus act as a “mother board” for self-assemblage of protein based “components”. Although producing DNA nanogrids has been the object of several publications in recent years [1.1-1.4], no attempt has been made so far to address single nanogrids onto specific locations of a planar surface, as required for device fabrication. In the present project, this will result from the long experience of the consortium in microelectronics oriented nanomachining [1.5], in coupling of biomolecules to solid state surfaces [1.6-1.8], in molecular biology [1.9-1.11] and DNA aptamers [1.12 – 1.14], and in general nanotechnology developments [1.15]. More specifically, the experts in DNA assemblage (NAST Centre, Roma) will design and produce the DNA planar grids, while the experts in DNA-protein interactions (Comenius University of Bratislava (CUB) will produce and test the proper aptamers for specific protein capture. The skills in atomic force microscopy and spectroscopy will be devoted to the characterization of the assembled structures (NAST, in collaboration with ENEA researchers, and CUB), while the capabilities in nanoelectronic device fabrication by electron-beam and focused ion beam lithography present at Oak Ridge National Laboratory/Centre for Nanophase Materials Science (CNMS-ORNL) will supply the addressable nanomachined inorganic structures on which the nanogrids will be positioned. Theory and computer modelling and simulation experts at both CNMS and NAST [1.6] will perform calculations relative to the optimization of adhesion of the grids to their supporting pads and to the optimization of the DNA-ligand interaction. The unique technology proposed will thus also allow preparing reproducible biomolecular capturing devices with high sensitivity and selectivity for a wide range of applications.
The idea of this wide ranging project was to supply some new technology advances based on integrated materials and methodologies which will pave the way to the exploitation of entirely new concepts in the development of micro and nanodevices, including those belonging to the branch of “nanomedicine”.
Workpackage 1, in particular, is based on the idea of exploiting the smart selfassembly properties of biomolecules to produce, arrange, localize and interface some type of structural scaffolds onto which molecular components will in turn selfassemble. This is in some way an attempt to mimick the way nature works, although we want, at this stage, to maintain the advantages offered by the velocity and the ruggedness of solid state devices. Thus, the project is devoted to fill in the gap that exists between the recently developed skills in producing artificially designed, but “naturally” selfassembling, nanoarchitectures based on DNA sequences, and the “user friendly” solid state electronics methods; these must necessarily be used to input and output signals from and to new envisageable “devices” based on soft matter.
The fundamental problem at the base of many such attempts is of course: in solution or in the dry, solid phase? Most biomolecular properties are at work in solution, where biomolecules are generally short lived in comparison to the life of solid state devices, so the issue may appear contradictory in itself. However, several examples of extremely stable organic or even biological macromolecular systems exist which retain a useful structure even with no water, which have functions in the solid phase, which have completely reversible structural transitions on switching from an acqueous to a dry environment, which are enormously more stable when suitably immobilized, and finally which display their useful properties with a molecularly thin hydration layer which does not induce fragmentation or severe structural changes. In particular, this latter issue deserves further and deeper investigation: how thick must the water layer around biomolecules to maintain their structural and functionl properties? This is clearly the object of computer simulation studies, some of which have been tackled in the course of this project.
At the present stage, the attempt of demonstrating the DNA motherboards concept (we would now rather describe it more generally as a DNA “breadboards” concept) aims at supplying the technologies to produce, orderly immobilize, and put at work, some smart architectures, based on DNA: these “nano-breadboards” will auto-assemble onto themselves, proteic components acting as sensing or electronically active elements.
One further interesting concept that has been explored in the course of the project is that of molecular electronic “connectors”; ordinary electronic boards connectors are characterized by a specific geometrical shape (that ensures that only the right components can be plugged in at their specific location) and by carrying one or more signals. DNA aptamers may be the analogous in selfassembling DNA breadboards for molecular electronics: indeeed aptamers can selectively bind specific proteins at well defined locations on the breadboard (+- 3 nm) and (unilke e.g. antibodies) are small enough to allow charge tunnelling to conductive components on the breadboard (e.g. conductive polymers, carbon nanotubes or graphene ribbons)
Objectives attained
What are the main advances obtained by our consortium along this direction?
We have obtained reliable ways of producing anchoring nanodots, via e-beam lithography in a very dependable and repetable fashion. In fact we have obtained features that are much smaller thanis needed, as we discovered, at our stage of development. Indeed, using nanodots around or even below the size of 10 nm is possible, but it doesn’t help in our demonstration work because the rate of DNA docking from the solution depends on the nanoanchors’ size, and it becomes really too low on such small structures. Increasing the size to 20-25 nm increases the docking rate by a factor 5 to 10 and it allows us to have statistically relevant numbers to analyze.
We have ascertained, also with the fundamental contributions of our collaborating partner from the Aarhus University, that “tiles” based, composable DNA nanostructures, are produced with a much lower and less reliable rate than DNA “origami” nanostructures. And that the origami structures are much more easily decorated or functionalized with “sticky ends” that we can use to hybridize other DNA based scaffolds or protein specific aptameric “connectors”.
We have demonstrated that such DNA origami slightly rectangular “breadboards” can be suspended across anchoring nanodots at a variable, and to some extent controllable, distance from the substrate, of the order of a few nanometers (up to 6-7 nm in our experiments so far).
This may have extremely important consequences for sensing and molecular electronics: a) it allow doubling of the number of components for each breadboard, which can be a double face one; b) it decouples the selfassembling mechanisms from the interaction with the substrate. It is thus a feature that deserves further dedicated attention. In fact we find that very often the narrow space between the suspended origami and the substrate is filled with precipitated nanocrystals from the buffer solutes, which are not easy to remove.
The space resolution with which we can locate our connectors is of the order of 5-6 nm, which means that we can have approximately one proteic component every approxiamtely 30 squared nanometers. For the type of 85×75 nm2 DNA origami that we use, this means approximately 200 components on each face of our “breadboard”. The drawback of DNA origami compact structures is on the other hand a higher non-specific adhesion to the substrate via van der Waals interactions.
Incubation of the origami solution has to proceed for many hours before a reasonable number of anchoring locations is covered by our nano-breadboards. Times as long as two days are necessary and a compromise must be seeked between the number of occupied locations and the disruption, or denaturation of the structures due to thermal fluctuations.
The order of magnitude of occupied locations after 45 hours of incubation is 10%. This may seem a disappointingly low figure, but in fact it is not if compared to other attempts that have been made recently with much larger DNA structures and nanoanchors. The problem lyes in the random way origami come in contact with the anchoring nanodots, and we expect the “docking” rate to be boosted by the next generation of “devices” in which suitably engineered connecting nanowires will allow for electrostatic attraction (dielectrophoresis) of the origami on theitr anchoring locations
We have also observed that the concentration of Mg counterions in the incubating solution is important to obtain an optimal compromise between a high rate of bonding on the gold nanostructures and a low rate of adsorption on the substrate. This concentration is around 70 100 micromolar. These details are described in depth in the report relative to deliverable D1.3.4
We have understood a number of issues related to the use of surface selective peptides for DNA immobilization but we have in the end only used sulphur gold bonding rather than the more difficult and laborious four level lithography to obtain four different materials nanodots for each device. This is because of two reasons: 1)the simulation was only reliably performed for Titania selective peptides. 2) we first need to have quantitative indications on the docking rates on the simplest system, which turn out to be still somewhat low, before we are able to study the more complex bonding directly on nanodots of different materials. On the other hand some of the orientational symmetries can be removed by use of strongly asymmetric origami, and of the consequent nanodots geometric arrangement, rather than by fabricating nanodots of four different materials.
Nevertheless peptides simulation supplied extremely important results on the most important selective adhesion processes, which are directly electrostatic. The activity on simulation of charge exchange processes on the other hand proceeded in the investigation of the most suitable computational methods to tackle such very complex multiscale problem. It was found that hybrid methodologies based on short range quantum chemistry DFT methods and molecular dynamics simulation on the longer range are the most convenient approaches both for the conformational changes of adhesive peptides and for conformationally modulated charge exchange which in the end is well described by the Marcus’ theory. Also reactive force field molecular dinamics methodologies represent a somewhat similar and parallel approach that describes well problems of conformational denaturation of macromolecules. The results of such investigation on available methodologies are described in depth in the report relative to D1.3.1
The observation of a strongly asymmetric conductance through helical peptides bound to gold was the most important result of the strong effort put in the experimental activity related to the spectroscopy of charge transfer across peptides in solution (D1.3.2). This led the NAST Center group of Professor Venanzi to obtain a wealth of results published in several papers. Such observations can have extremely valuable consequences in the achievement of specific molecular electronic devices selfassembled on DNA based scaffolds.
The experimental activity carried out at Oak Ridge in collaboration with the Bratislava group and the NAST Center researchers led to progresses in the detection of docking events of proteins to gold bonded aptamers and of DNA origami on gold nanopillars. A cell for simultaneous detection of the dockingevents via SERS and QCM was developed and is now in use. The protein Thrombin was successfully bound to its aptamer and to tridimensional selfassembled scaffolds in the shape of a tetrahedron (Deliverable 1.3.3 1.3.4 and 1.3.5).
Thus the scheduled results have been obtained, and we have now very solid ground on which the development of this idea can be further built. The final experiment of measuring electrical currents through nano”motherboards” assempled molecular components is also completed.
Collaboration and results obtained within META have driven a newly submitted project (CONORI project) within the Future and Emerging Technologies scheme (under evaluation). Having attracted the interest of more outstanding research groups on the subject, the CONORI project has brough together the META partners of WP1 and additional partners from Denmark, namely the groups of Professor Kurt Gothelf at Aarhus and of Professor Anja Boisen from the Technical University of Kobenhavn and the Molecular Electronics group of Professor Danny Porath at the Hebrew University of Jerusalem.
List of references
[1.1] N. C. Seeman and, A. M. Belcher; Emulating biology: building nanostructures from the bottom up. PNAS 2002, 99,6451–6455
[1.2] H. Li, J. D. Carterand, T. H. LaBean; Nanofabrication by DNA selfassembly. Materials Today 2009,12, 24
[1.3] S. Ha Park, C. Pistol, Sang, J. Ahn, J. H. Reif, A. R. Lebeck, C. Dwyer, T. H. LaBean; Finite-Size, Fully Addressable DNA Tile Lattices Formed by Hierarchical Assembly Procedures. Angew. Chem. Int. Ed. 2006, 45, 735 –739
[1.4] H. Li,T. H. LaBean, D. J. Kenan; Single-chain antibodies against DNA aptamers for use as adapter moleculeson DNA tile arrays in nanoscale materials organization. Org. Biomol. Chem. 2006, 4, 3420–3426
[1.5] A. Gerardino, A. Notargiacomo, P. Morales, Laser assisted deposition of nanopatterned biomolecular layers. Microelectronic Engineering 2003, 67-8, 923-929
[1.6] G. Gianese, V. Rosato, F. Cleri, M. Celino, P. Morales; Atomic-Scale Modeling of the Interaction between Short Polypeptides and Carbon Surfaces. J. Phys. Chem. B 2009, 113, 12105–12112; S. Gagliardi, B. Rapone, L. Mosiello, D. Luciani, A. Gerardino, P. Morales; Laser-Assisted Fabrication of Biomolecular Sensing Microarrays. IEEE Transactions on Nanobioscience, 2007, 6(3), 242 – 248; Ulmschneider, Martin B., Doux, Jacques P. F. Killian, J. Antoinette, Smith, Jeremy C., Ulmschneider, Jakob P., Mechanism and Kinetics of Peptide Partitioning into Membranes from All-Atom Simulations of Thermostable Peptides; J. Am. Chem. Soc. 2010, 132, 3452; Sumpter, B.G., Getino, C., Noid D.W.; Computational Studies of Submicron Probing of Polymer Surfaces; J.Chem. Phys. 96,7072 (1992)
[1.7] E. Gatto, L. Stella, F. Formaggio, C. Toniolo, L. Lorenzelli, M. Venanzi; Electro-conductive and photocurrent generation properties of self-assembled monolayers formed by functionalized, conformationally-constrained peptides on gold electrodes; J. Pept. Sci. 2008, 14, 184-191
[1.8] M. Venanzi, G. Pace, A. Palleschi, L. Stella, P. Castrucci, M. Scarselli, M. De Crescenzi, F. Formaggio, C. Toniolo, G. Marletta; Densely-packed self-assembled monolayers on gold surfaces from a conformationally constrained helical hexapeptide. Surf. Sci. 2006, 600, 409-416
[1.9] R. Biondo, A. Caglià, P. Asili, G. D’Agostaro, G. D’Agostino, A. Spinella; Typing of 20 Y-chromosome STRs in the Italian population. Forensic Sci Int. 2004,146 Suppl:S135-138
[1.10] G. A. D’Agostaro, A. Zingoni, R. L. Moritz, R. J. Simpson, H. Schachter, B. Bendiak; Molecular cloning and expression of cDNA encoding the rat UDP-N-acetylglucosamine: a6-D-mannoside β-1,2-N-acetylglucosaminyltransferase II. J. Biol. Chem. 1995, 270(25),15211-15221
[1.11] G. D’Agostaro, E. Hevia, G. E. Wu, H. Murialdo; Site-directed cleavage of immunoglobulin gene segments by lymphoid cell extracts. Can. J. Biochem. Cell Biol. 1985 63(9), 969-976.
[1.12] T. Hianik, I. Grman, I. Karpisova; The effect of DNA aptamer configuration on the sensitivity of detection thrombin at surface by acoustic method. Chem. Commun. 2009, 6303–6305.
[1.13] T. Hianik, A. Porfireva, I. Grman, G. Evtugyn; EQCM biosensors based on DNA aptamers and antibodies for rapid detection of prions. Protein and Peptide Letters, 2009, 16, 363-367
Description of work
WP1 will be organized into three tasks:
Task 1: Self-assemblage of single streptavidine molecules in preprogrammed locations of a nanopositioned DNA grid interfaced to nanomachined inorganic pads
The NAST/ENEA and the CUB groups will initially produce DNA grids programmed to react with only one type of protein (streptavidin) in two or three preprogrammed grid crossings, and also endowed with material-selective adhesive peptides at their corners to achieve adhesion onto the different pads materials, and consequently orientationally controlled immobilization of the whole grid (see fig. 1). In the early stage of the project a simpler prototype will also be prepared, consisting of a similar DNA grid, functionalized at the four corners with four identical oligopeptide arms. These peptide chains will comprise exclusively Cα-tetrasubstitutedaminoacids, that endow the peptide with rigid conformational properties. Such peptidicnanopillars, designed to separate the grid from the substrate, will be functionalized at the N-terminus by lipoic acid, a moiety containing a disulfide group that will bind the nanogrid-peptides complex to four gold nanopads. CNMS lithography capabilities will be used to produce electrically connected pads of gold as well as of different materials designed to immobilize the grids in addressable locations, with recognizable patterns (e.g. regularly spaced groups of a few tens of grids that could be easily identified by available characterization techniques, e.g., fluorescence microscopy). The PLD thin layer deposition can be effected at NAST and/or CNMS. The NAST group shall then attempt a first immobilization of the biomaterial on the inorganic chip, analyze the results by AFM and fluorescence microscopy, checking the successful docking of streptavidin molecules in the pre-programmed locations of the grids.
This part of the work is based on previously well assessed and published work performed within the consortium and in other laboratories and on well established state of the art technologies. Therefore we simply aim to joining forces in single already established steps to obtain a new advancement in technology.
Efficient immobilization of the DNA nanogrids requires modelling of the peptide – inorganic surface interaction in order to optimize selective adhesion and to decide if a DNA-surface selective peptide spacer is necessary and of what size. We need to understand if and how the peptide conformation, and its consequent adhesion on the surface, is modified by the attachment to the edge “arm” of the DNA nanogrid. This can be tackled as a user project by the theory group at CNMS in collaboration with the interested NAST researchers on the basis of the discussions within the first two joint meetings. Modelling approaches will focus on relative strength of binding interactions among the inorganic surface, DNA, and protein moieties of selected model systems. Appropriate systems will be identified based on suggestions from consortium molecular biologists. To accurately guide the selection of the optimal peptide to attach DNA “mother boards” to the appropriate substrates, CNMS will use first principles quantum density functional theory (DFT) exploring the fundamental interactions between a small peptide and the specified substrates. The DFT study will take into account non-local contributions to the exchange-correlation functional to determine contributions to the underlying van der Waals interactions. The effects of a solvent medium will be accounted for by using a reaction field model. A similar approach can also be used to examine the fundamental interaction between aptamers and small proteins. Dynamical effects and thermal stability will be examined by using molecular dynamics (MD), either via quantum MD or by using the information obtained from the DFT study.
The next consortium meetings should also start paving the way to the second task of the project, where we would like to employ a larger number of proteins recognizing and sticking to specific DNA sequences in the mesh.
Task 2: Self-assemblage of different protein molecules in preprogrammed locations of a nanopositioned DNA grid interfaced to nanomachined inorganic pads
Task 2 will proceed as follows: Modelling and dynamical simulation of a selection of protein DNA sequence interaction, including naturally occurring ones and artificially selected aptamers. Choice of the best candidates. Engineering and assembling new DNA meshes including the chosen sequences or aptamers developed at CUB. Specific protein binding and test. Possibly, more lithography work for immobilization of the new grids. Immobilization of the engineered grid onto the lithographic pattern. Selfassemblage of the selected protein molecules onto their target DNA sites. Characterization of the selfassembled proteic components by spectroscopy and scanning probe microscopy.
Task 3 Use of the addressable nanoscopic “mother boards” for the self-assemblage of functional devices
Task 3 will be devoted to the exploitation of the DNA planar architecture (the “mother board”) and of the protein-DNA specific docking process to self-assemble specific devices. On the basis of the milestones reached by the previous tasks, these will be a charge exchange system and/or a DNA fragment capture system that can be exploited for hybridization of longer DNA stretches that can be detected and imaged by AFM.
Research team
CUB: Professor T. Hianik, Dr M. Snejdarkova, Dr P. Rybar, Ph.D. Students
NAST: Dr C. Arcangeli, Dr S. Baschieri, Dr Ugo Borriello, Dr C. Cantale, Dr M. Celino, Dr G. D’Agostaro, Dr C. Dalmastri, Prof A. Desideri, Dr C. Lico, Dr P. Morales, Dr L. Mosiello, Dr. L. Pilloni, Prof M. Venanzi; Postdoctoral Students and Fellows: Mario Caruso, Nicola Pellicciotta, Stefano Vespucci, Liqian Wang
ORNL: Dr J. M. Simonson, Dr S. T. Retterer, Dr B. G. Sumpter, Dr M. Fuentes-Cabrera, Dr P. R. Kent, Dr. I.Ivanov, Dr. Patrick Collier, Dr. Alex Puretzky
————————
ALLEGATO I